Introduction
- Heart-brain Communication
- Heart Rate Variability: An Indicator of Self-Regulatory Capacity, Autonomic Function and Health
- Coherence
- Establishing a New Baseline
- Bibliography
Introduction
The HeartMath® Institute Research Center has explored the physiological mechanisms by which the heart and brain communicate and how the activity of the heart influences our perceptions, emotions, intuition and health. In the early 1990s, we were among the first to conduct research that not only looked at how stressful emotions affect the activity in the autonomic nervous system and the hormonal and immune systems, but also at the effects of emotions such as appreciation, compassion and care. Over the years, we have conducted many studies that have utilized many different physiological measures such as EEG (brain waves), SCL (skin conductance), ECG (heart), BP (blood pressure) and hormone levels, etc. Consistently, however, it was heart rate variability, or heart rhythms that stood out as the most dynamic and reflective indicator of one’s emotional states and autonomic nervous system dynamics. It became clear that stressful or depleting emotions such as frustration and overwhelm lead to increased disorder in the higher-level brain centers which is reflected in heart rhythms and adversely affects the functioning of virtually all bodily systems. This eventually led to a much deeper understanding of the neural and other communication pathways between the heart and brain. Numerous studies have since shown that heart coherence is an optimal state-specific physiological pattern associated with increased cognitive function, self-regulatory capacity, emotional stability and resilience, and that people can learn to shift into the coherent state in the heat of the moment.
Our research and that of others indicates the heart is far more than a simple pump. The heart is, in fact, a highly complex information processing center with its own functional “brain,” commonly called the heart-brain that communicates with and influences the cranial brain via the nervous system, hormonal system and other pathways. These influences profoundly affect brain function and most of the body’s major organs and play an important role in mental and emotional experience and the quality of our lives. Healthy, optimal function is a result of continuous, dynamic, bi-directional interactions among multiple neural, hormonal and mechanical control systems at both local and central levels. In concert, these dynamic interconnected physiological and psychological regulatory systems are never truly at rest and are certainly never static. For example, we now know that the normal resting rhythm of the heart is highly variable rather than monotonously regular, which was the widespread notion for many years. This will be discussed further in the section on heart rate variability (HRV).
Heart-Brain Communication
Traditionally, the study of communication pathways between the “head” and heart has been approached from a rather one-sided perspective, with the focus being primarily on the heart’s responses to the brain’s commands. However, the communication between the heart and brain actually is a dynamic, ongoing, two-way dialogue, with each organ continuously influencing the other’s function. Research has shown that the heart communicates to the brain in four major ways: neurologically (through the transmission of nerve impulses), biochemically (via hormones and neurotransmitters), biophysically (through pressure waves) and energetically (through electromagnetic field interactions). Communication along all these conduits significantly affects the brain’s activity and our performance.
Neurocardiology: The Heart Brain
The anatomy and functions of the intrinsic cardiac nervous system and its connections with the brain have been explored extensively in the field of neurocardiology.[1, 2] In terms of heart-brain communication, it is generally well-known that the efferent (descending) pathways in the autonomic nervous system (ANS) are involved in the regulation of the heart. However, it is less appreciated that the majority of fibers in the vagus nerves are afferent (ascending) in nature. Furthermore, more of these ascending neural pathways are related to the heart (and cardiovascular system) than to any other organ.[3] This means the heart sends more information to the brain than the brain sends to the heart. More recent research shows that the neural interactions between the heart and brain are more complex than previously thought. In addition, the intrinsic cardiac nervous system has both short-term and long-term memory functions and can operate independently of central neuronal command.
Once information has been processed by the heart’s intrinsic nervous system, the appropriate signals are sent to the heart’s sinoatrial node and to other tissues in the heart. Thus, under normal physiological conditions, the heart’s intrinsic nervous system plays an important role in much of the routine control of cardiac function, independent of the central nervous system. The heart’s intrinsic nervous system is vital for the maintenance of cardiovascular stability and efficiency and without it, the heart cannot function properly. The neural output or “messages” from the intrinsic cardiac nervous system travels to the brain via ascending pathways in the both the spinal column and vagus nerves, where it travels to the medulla, hypothalamus, thalamus and amygdala and then to the cerebral cortex.[4-6] The nervous system pathways between the heart and brain are shown in Figure 1 and the primary afferent pathways in the brain are shown in Figure 2.
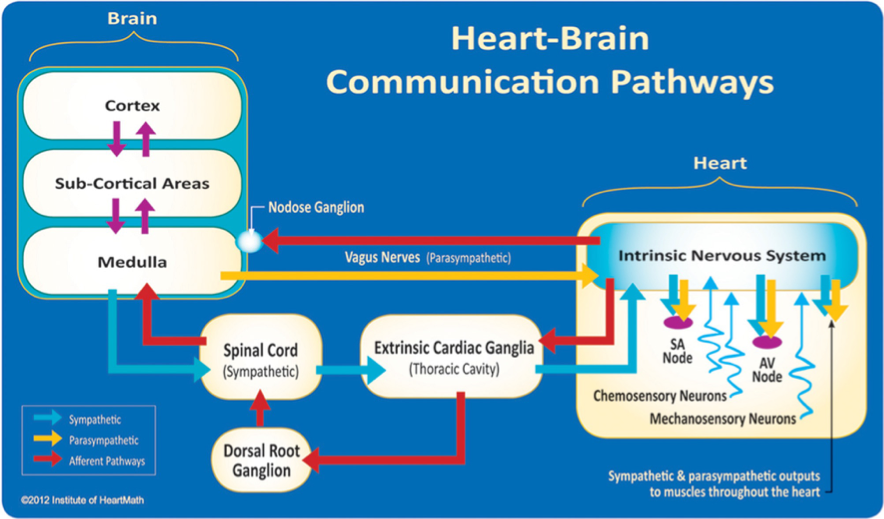
Figure 1. The neural communication pathways interacting between the heart and the brain are responsible for the generation of HRV. The intrinsic cardiac nervous system integrates information from the extrinsic nervous system and from the sensory neurites within the heart. The extrinsic cardiac ganglia located in the thoracic cavity have connections to the lungs and esophagus and are indirectly connected via the spinal cord to many other organs, including the skin and arteries. The vagus nerve (parasympathetic) primarily consists of afferent (flowing to the brain) fibers that connect to the medulla. The extrinsic cardiac ganglia, located in the thoracic cavity, have direct connections to organs such as the lungs and esophagus and also are indirectly connected via the spinal cord to many other organs, including the skin and arteries. The sympathetic afferent nerves first connect to the extrinsic cardiac ganglia (also a processing center), then to the dorsal root ganglion and the spinal cord. Once afferent signals reach the medulla, they travel to the subcortical areas (thalamus, amygdala, etc.) and then to the higher cortical areas.
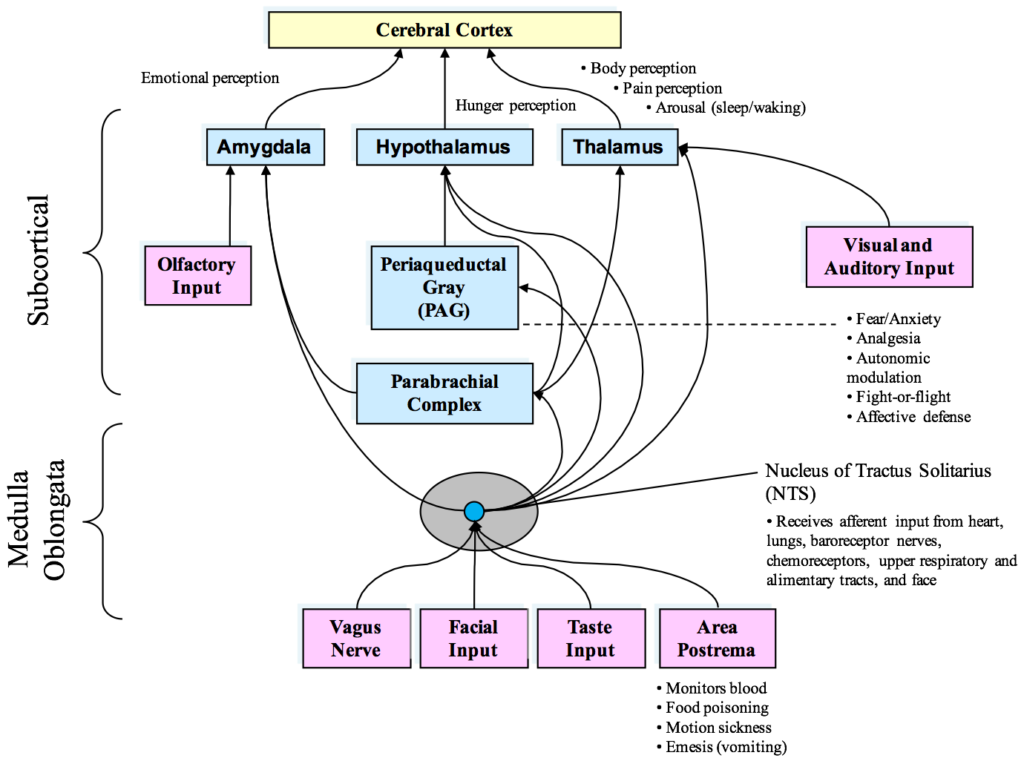
Figure 2. Diagram of the currently known afferent pathways by which information from the heart and cardiovascular system modulates brain activity. Note the direct connections from the NTS to the amygdala, hypothalamus and thalamus. Although not shown, there also is evidence emerging that there is a pathway from the dorsal vagal complex that travels directly to the frontal cortex
Heart Rate Variability: An Indicator of Self-Regulatory Capacity, Autonomic Function and Health
The autonomic nervous system (ANS) (Figure 3) is the part of the nervous system that controls the body’s internal functions, including heart rate, gastrointestinal tract and secretions of many glands. The ANS also controls many other vital activities such as respiration and it interacts with immune and hormonal system functions. It is well known that mental and emotional states directly affect activity in the ANS.
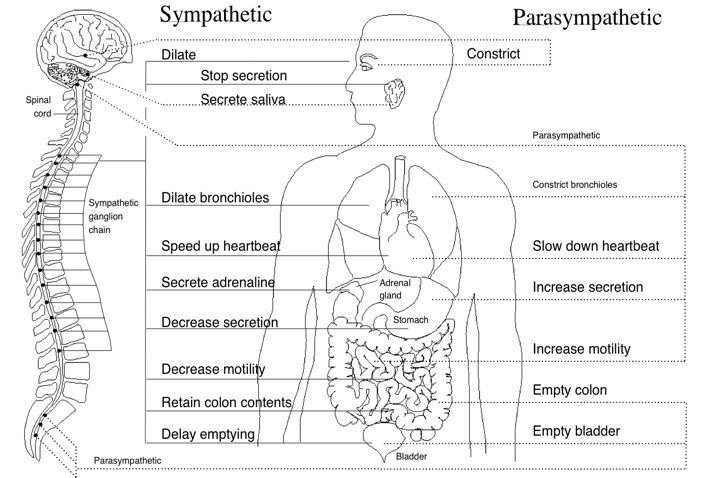
Figure 3. Innervation of the major organs by the autonomic nervous system (ANS). Parasympathetic fibers are primarily in the vagus nerves, but some that regulate subdiaphragmatic organs travel through the spinal cord. The sympathetic fibers also travel through the spinal cord. A number of health problems can arise in part because of improper function of the ANS. Emotions can affect activity in both branches of the ANS. For example, anger causes increased sympathetic activity while many relaxation techniques increase parasympathetic activity.
The autonomic nervous system must be considered as a complex system in which both efferent (descending) and afferent (ascending) neurons regulate adaptive responses. Considerable evidence suggests evolution of the ANS, specifically the vagus nerves, was central to development of emotional experience, the ability to self-regulate emotional processes and social behavior and that it underlies the social engagement system. As human beings, we are not limited to fight, flight, or freeze responses. We can self-regulate and initiate pro-social behaviors when we encounter challenges, disagreements and stressors. The healthy function of the social engagement system depends upon the proper functioning of the vagus nerves, which act as a “vagal brake.” This system underlies one’s ability to self-regulate and calm oneself by inhibiting sympathetic outflow to targets like the heart and adrenal glands. This implies that measurements of vagal activity could serve as a marker for one’s ability to self-regulate. This also suggests that the evolution and healthy function of the ANS determines the boundaries for the range of one’s emotional expression, quality of communication and the ability to self-regulate emotions and behaviors.[7]
The investigation of the heart’s complex rhythms, or HRV began with the emergence of modern signal processing in the 1960s and 1970s and has rapidly expanded in more recent times.[8] The irregular behavior of the heartbeat is readily apparent when heart rate is examined on a beat-to-beat basis, but is overlooked when a mean value over time is calculated. These fluctuations in heart rate result from complex, nonlinear interactions among a number of different physiological systems.
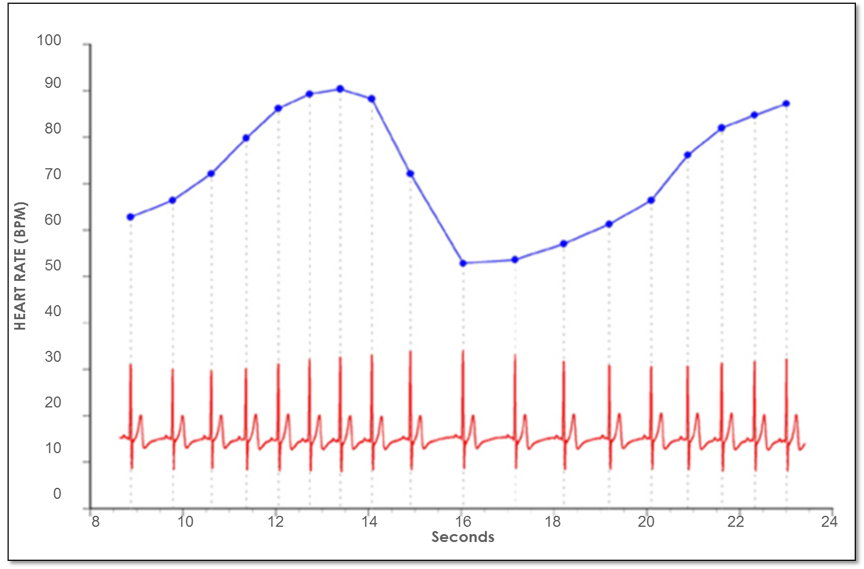
Figure 4. Heart rate variability is a measure of the normally occurring beat-to-beat changes in heart rate. The electrocardiogram (ECG) is shown on the bottom and the instantaneous heart rate is shown by the blue line. The time between each of the heartbeats (blue line) between 0 and approximately 13 seconds becomes progressively shorter and heart rate accelerates and then starts to decelerate around 13 seconds. This pattern of heart-rate accelerations and decelerations is the basis of the heart’s rhythms.
An optimal level of HRV within an organism reflects healthy function and an inherent self-regulatory capacity, adaptability, and resilience.[5, 9-14] While too much instability, such as arrhythmias or nervous system chaos, is detrimental to efficient physiological functioning and energy utilization, too little variation indicates age-related system depletion, chronic stress, pathology or inadequate functioning in various levels of self-regulatory control systems.[8, 15, 16]
The importance of HRV as an index of the functional status of physiological control systems was noted as far back as 1965, when it was found that fetal distress was preceded by reductions in HRV before any changes occur in heart rate itself.[17] In the 1970s, reduced HRV was shown to predict autonomic neuropathy in diabetic patients before the onset of symptoms.[18-20] Reduced HRV also was found to be a higher risk factor of death post-myocardial infarction than other known risk factors.[21] It has been shown that HRV declines with age and that age-adjusted values should be used in the context of risk prediction.[22] Age-adjusted HRV that is low has been confirmed as a strong, independent predictor of future health problems in both healthy people and in patients with known coronary artery disease and correlates with all-cause mortality.[23, 24]
Based on indirect evidence, reduced HRV may correlate with disease and mortality because it reflects reduced regulatory capacity and ability to adapt/respond to physiological challenges such as exercise. For example, in the Chicago Health, Aging and Social Relations Study, separate metrics for the assessment of autonomic balance and overall cardiac autonomic regulation were developed and tested in a sample of 229 participants. In this study, overall regulatory capacity was a significant predictor of overall health status, but autonomic balance was not. In addition, cardiac regulatory capacity was negatively associated with the prior incidence of myocardial infarctions. The authors suggest that cardiac regulatory capacity reflects a physiological state that is more relevant to health than the independent sympathetic or parasympathetic controls, or the autonomic balance between these controls as indexed by different measures of HRV.[25]
Heart rate variability also indicates psychological resiliency and behavioral flexibility, reflecting an individual’s capacity to self-regulate and effectively adapt to changing social or environmental demands.[25, 26] A growing number of studies have specifically linked vagally mediated HRV to self-regulatory capacity,[13, 14, 27] emotional regulation,[28, 29] social interactions,[11, 30] one’s sense of coherence[31] and the personality character traits of self-directedness[32] and coping styles.[33] More recently, several studies have shown an association between higher levels of resting HRV and performance on cognitive performance tasks requiring the use of executive functions.[15] HRV coherence (described later) can be increased in order to improve cognitive function[5, 34-36] as well as a wide range of clinical outcomes, including reduced health-care costs.[9, 37-42]
Self-Regulation: Cortical Systems
Considerable evidence from clinical, physiological and anatomical research has identified cortical, subcortical and medulla oblongata structures involved in cardiac regulation. Oppenheimer and Hopkins mapped a detailed hierarchy of cardiac control structures among the cortex, amygdala and other subcortical structures, all of which can modify cardiovascular-related neurons in the lower levels of the neuraxis (Figure 5).[43] They suggest that the amygdala is involved with refined integration of emotional content in higher centers to produce cardiovascular responses that are appropriate for the emotional aspects of the current circumstances. The insular cortex and other centers such as the orbitofrontal cortex and cingulate gyrus can overcome (self-regulate) emotionally entrained responses by inhibiting or enhancing them. They also point out that imbalances between the neurons in the insula, amygdala and hypothalamus may initiate cardiac rhythm disturbances and arrhythmias. The data suggests that the insular and medial prefrontal cortexes are key sites involved in modulating the heart’s rhythm, particularly during emotionally charged circumstances.
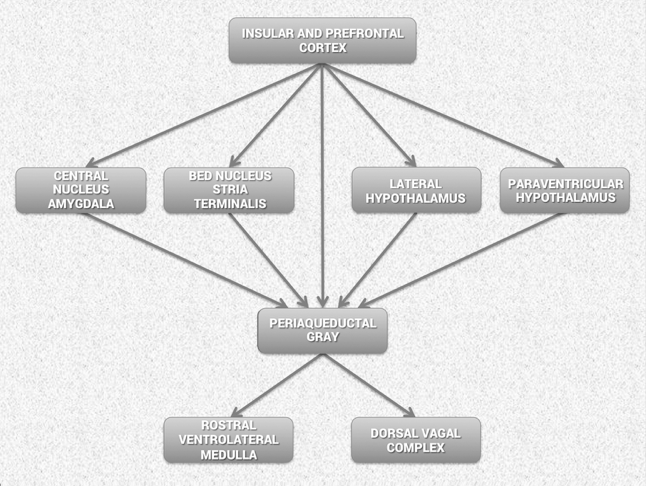
Figure 5. Schematic diagram showing the relationship of the principal descending neural pathways from the insular and prefrontal cortex to subcortical structures and the medulla oblongata as outlined by Oppenheimer and Hopkins.[43] The insular and prefrontal cortexes are key sites involved in modulating the heart’s rhythm, particularly during emotionally charged circumstances. These structures alone with other centers such as the orbitofrontal cortex and cingulate gyrus can inhibit or enhance emotional responses. The amygdala is involved with refined integration of emotional content in higher centers to produce cardiovascular responses that are appropriate for the emotional aspects of “current” circumstances. Imbalances between the neurons in the insula, amygdala and hypothalamus may initiate cardiac rhythm disturbances and arrhythmias. The structures in the medulla represent an interface between incoming afferent information from the heart, lungs and other bodily systems and outgoing efferent neuronal activity.[43]
Thayer and Lane also have described the same set of neural structures outlined by Oppenheimer and Hopkins, which they call the central autonomic network (CAN). The CAN is involved in cognitive, emotional and autonomic regulation, which they linked directly to HRV and cognitive performance. In their model, the CAN links the nucleus of tracus solitarius in the medulla with the insula, prefrontal cortex, amygdala and hypothalamus through a series of feedback and feed-forward loops. They also propose that this network is an integrated system for internal self-regulation by which the brain controls the heart and other internal organs, neuroendocrine and behavioral responses that are critical for goal-directed behavior, adaptability and sustained health. They suggest that these dynamic connections explain why parasympathetically (vagal) mediated HRV is linked to higher-level executive functions and reflects the functional capacity of the brain structures that support working memory and emotional and physiological self-regulation. They have shown that higher levels of vagally mediated HRV are correlated with prefrontal cortical performance and the ability to inhibit unwanted memories and intrusive thoughts. The prefrontal cortex can be taken “offline” when individuals perceive that they are threatened and prolonged periods of prefrontal cortical inactivity can lead to hypervigilance, defensiveness and social isolation. During these decreases in prefrontal cortical activation, HR increases and HRV decreases.[15]
The nucleus of tracus in the medulla oblongata integrates afferent sensory information from proprioceptors (body position), chemoreceptors (blood chemistry) and mechanoreceptors, also called baroreceptors, (pressure or distortion) from the heart, lungs and face. The nucleus of tracus connects to the dorsal motor nucleus of the vagus nerve and the nucleus ambiguous. Neurocardiology research indicates that the descending vagal fibers that innervate the heart are primarily A-fibers, which are the largest and fastest conducting axons that originate from nerve cells located primarily in the nucleus ambiguus. The nucleus ambiguus also receives and integrates information from the cortical and subcortical systems described above.[44] Thus, the vagal regulatory centers respond to peripheral sensory (afferent) inputs and higher brain-center inputs to adjust neuronal outflows, which results in the vagally mediated beat-to-beat changes in HR.
Increased efferent activity in the vagal nerves (also called the 10th cranial nerve) slows HR and increases bronchial tone. The vagus nerves are the primary nerves for the parasympathetic system and they innervate the intrinsic cardiac nervous system. A few of these connections synapse on motor neurons in the intrinsic cardiac nervous system and these neurons project directly to the SA node (and other tissues in the heart), where they trigger acetylcholine release to slow HR.[45] However, the majority of the efferent preganglionic vagal neurons (~80%) connect to local circuitry neurons in the intrinsic cardiac nervous system, where motor information is integrated with inputs from mechanosensory and chemosensory neurons in the heart.[46] Thus, efferent sympathetic and parasympathetic activity is integrated in and with the activity occurring in the heart’s intrinsic nervous system, including the input signals from the mechanosensory and chemosensory neurons within the heart, all of which ultimately contribute to beat-to-beat cardiac functional changes.[6]
In summary, the cardiorespiratory control system is complex and information from many inputs is integrated at multiple levels of the system, all of which are important for the generation of normal beat-to-beat variability in HR and BP. The medulla oblongata is the major structure integrating incoming afferent information from the heart, lungs and face with inputs from cortical and subcortical structures and is the source of the respiratory modulation of the activity patterns in sympathetic and parasympathetic outflow. The intrinsic cardiac nervous system integrates mechanosensitive and chemosensitive neuron inputs with efferent information from both the sympathetic and parasympathetic inputs from the brain, and as a complete system affects HRV, vasoconstriction and cardiac contractility in order to regulate HR and blood pressure.[47]
HRV and Analysis Methods
The normal variability in heart rate results from the descending (efferent) and the ascending (afferent) activity occurring in the two branches of the ANS, which act in concert, along with mechanical, hormonal and other physiological mechanisms to maintain cardiovascular parameters in their optimal ranges and to permit appropriate adjustments to changing external and internal conditions and challenges.
At rest, both sympathetic and parasympathetic nerves are tonically active, with the vagal effects predominant. Therefore, heart rate best reflects the relative balance between the sympathetic and parasympathetic systems. When speaking of autonomic balance, it should be kept in mind that a healthy system is constantly and dynamically changing. Therefore, an important indicator of the health status of the regulatory systems is that they have the capacity to respond to and adjust the relative autonomic balance, as reflected in heart rate, to the appropriate state for whatever a person is engaged in at any given moment. In other words, does the HR dynamically respond and is it higher in the daytime or when dealing with challenging tasks and lower when at rest or during sleep? An inability of the physiological self-regulatory systems to adapt to the current context and situation is associated with numerous clinical conditions.[48] Also distinct, altered, circadian patterns in 24-hour heart rates are associated with different and specific psychiatric disorders, particularly during sleep.[49, 50]
Heart rate estimated at any given time represents the net effect of the neural output of the parasympathetic (vagus) nerves, which slow HR and the sympathetic nerves, which accelerate it. In a denervated human heart where there are no connections from the ANS to the heart following its transplantation, the intrinsic rate generated by a pacemaker (SA node) is about 100 BPM.[51] Parasympathetic activity predominates when HR is below this intrinsic rate during normal daily activities and when at rest or sleep. When HR is above ~100 BPM, the relative balance shifts and sympathetic activity predominates. The average 24-hour HR in healthy people is ~73 BPM. Higher HRs are independent markers of mortality in a wide spectrum of conditions.[48]
It is important to note the natural relationship between HR and amount of HRV. As HR increases there is less time between heartbeats for variability to occur, so HRV decreases, while at lower HRs there is more time between heartbeats, so variability naturally increases. This is called cycle length dependence, and it persists in the healthy elderly to a variable degree, even at very advanced ages. However, elderly patients with ischemic heart disease or other pathologies increasingly have less variability as HRs decrease, ultimately losing the relationship between HR and variability — to the point that variability does not increase at all with reductions in HR.[52] Even in healthy subjects, the effects of cycle length dependence should be taken into account when assessing HRV, and HR values should always be reported, especially when HRs are increased because of factors such as stress reactions, medications and physical activity.
An increase in sympathetic activity is the principal method used to increase HR above the intrinsic level generated by the SA node. Activation of this branch of the ANS, in concert with the activation of the endocrine system, facilitates the ability to respond to challenges, stressors or threats by increasing the mobilization of energy resources.
Following the onset of sympathetic stimulation, there is a delay of up to 5 seconds before the stimulation induces a progressive increase in HR, which reaches a steady level in 20 to 30 seconds if the stimulus is continuous.[47] The relatively slow response to sympathetic stimulation is in direct contrast to vagal stimulation, which is almost instantaneous. However, the effect of sympathetic stimulation on HR is longer-lasting and even a brief stimulus can affect HR for 5 to 10 seconds. Efferent (descending) sympathetic nerves target the SA node via the intrinsic cardiac nervous system and the bulk of the myocardium (heart muscle). Action potentials conducted by these motor neurons trigger norepinephrine and epinephrine release, which increases HR and strengthens the contractility of the atria and ventricles.
HRV can be assessed with various analytical approaches, although the most commonly used are frequency domain (power spectral density) analysis and time domain analysis. In both methods, the time intervals between each successive normal QRS complex are first determined. All abnormal beats not generated by the sinus node are eliminated from the record. The interactions between autonomic neural activity, BP, respiratory and higher level control systems produce both short- and longer-term rhythms in HRV measurements.[5, 53, 54] The most common form for observing these changes is the heart-rate tachogram, a plot of the sequence of time intervals between heartbeats (Figure 6).
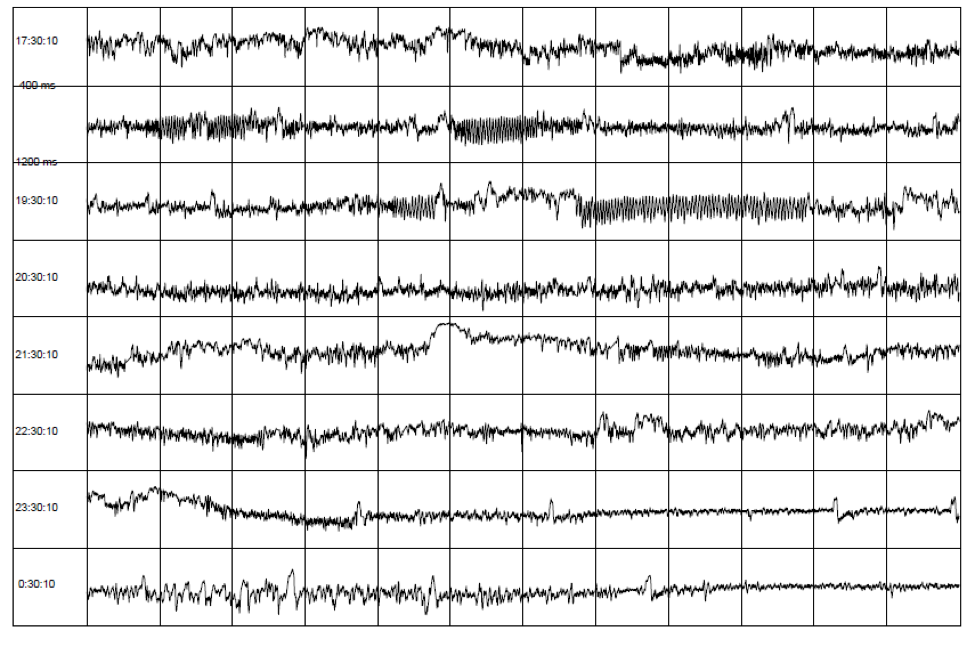
Figure 6. An example of the heart-rate tachogram, a plot of the sequence of time intervals between heartbeats over an 8-hour period in ambulatory recording taken from a 36-year-old male. Each of the traces is one hour long, with the starting time of the hour on the left-hand side of the figure. The time between each vertical line is 5 minutes. The vertical axis within each of the hourly tracings is the time between heartbeats (inter beat intervals) ranging from 400 and 1,200 milliseconds (label shown on second row). A 15-minute period of HRV coherence can be seen in the latter part of the hour, starting at 19:30 when this man used HeartMath’s Heart Lock-In® Technique. The latter part of the hour, starting at 23:30, is typical of restful sleep.
Power spectral analysis is used to separate the complex HRV waveform, into its component rhythms (Figure 7). Spectral analysis provides information about how power is distributed (the variance and amplitude of a given rhythm) as a function of frequency (the time period of a given rhythm). The main advantages of spectral analysis over the time domain measures are that it supplies both frequency and amplitude information on the specific rhythms that exist in the HRV waveform, providing a means to quantify these oscillations over any given period. The values are expressed as the power spectral density, which is the area under the curve (peak) in a given bandwidth of the spectrum. The power or height of the peak at any given frequency indicates the amplitude and stability of the rhythm. The frequency reflects the period of time over which the rhythm occurs. For example, a 0.1 hertz frequency has a period of 10 seconds. In order to understand how power spectral analysis distinguishes the various underlying physiological mechanisms reflected in the heart’s rhythm, a brief discussion of the underlying physiological mechanisms is helpful.
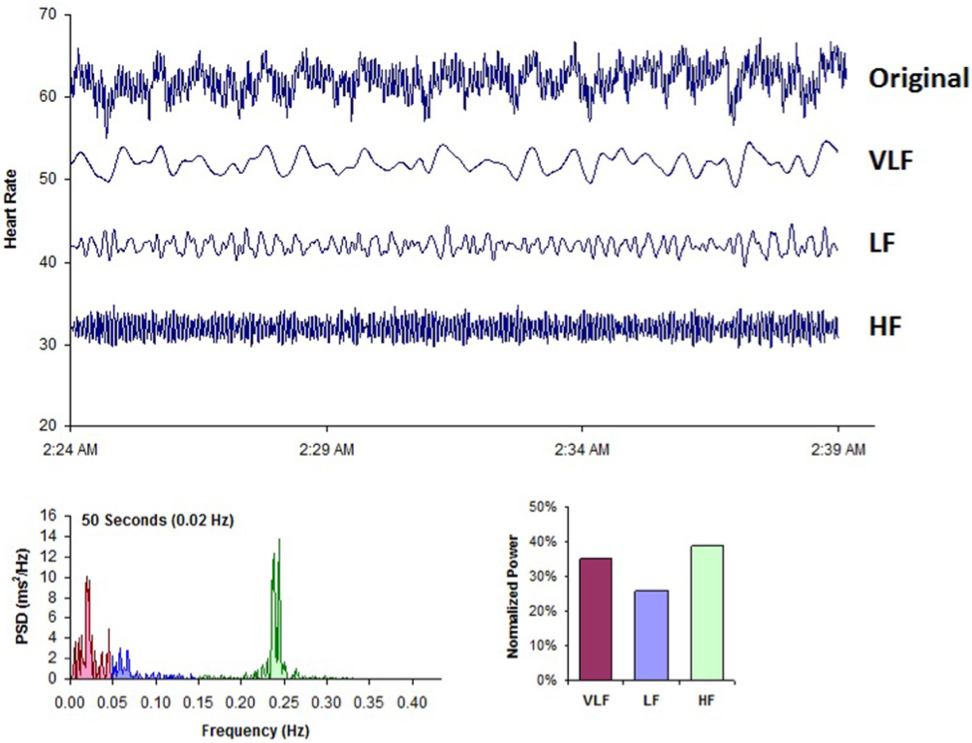
Figure 7. This figure shows a typical HRV recording over a 15-minute period during resting conditions in a healthy individual. The top trace shows the original HRV waveform. Filtering techniques were used to separate the original waveform into VLF, LF, and HF bands as shown in the lower traces. The bottom of the figure shows the power spectra (left) and the percentage of power (right) in each band.
The power spectrum is divided into three main frequency ranges.
High-Frequency Band
The high-frequency (HF) spectrum is the power in the range from 0.15 to 0.4 hertz, which equates to rhythms with periods that occur between 2.5 and 7 seconds. This band reflects parasympathetic or vagal activity and is frequently called the respiratory band because it corresponds to the HR variations related to the respiratory cycle known as respiratory sinus arrhythmia. The mechanisms linking the variability of HR to respiration are complex and involve both central and reflex interactions.[44] During inhalation, the cardiorespiratory center inhibits vagal outflow, resulting in speeding up HR. Conversely, during exhalation, vagal outflow is restored, resulting in slowing HR.[55] The magnitude of the oscillation is variable, but in healthy people, it can be increased by slow, deep breathing. Reduced parasympathetic (HF) activity has been found in a number of cardiac pathologies as discussed earlier. In terms of psychological regulation, reduced vagally mediated HRV has been linked to reduced self-regulatory capacity and cognitive functions that involve the executive centers of the prefrontal cortex. This is consistent with the finding that lower HF power is associated with stress, panic and anxiety/worry. Lower parasympathetic activity, rather than reduced sympathetic functioning, appears to account for a higher ratio of the reduced HRV in aging.[22]
Low-Frequency Band
The low-frequency (LF) band ranges between 0.04 and 0.15 hertz, which equates to rhythms or modulations with periods that occur between 7 and 25 seconds. This region was previously called the “baroreceptor range” or “midfrequency band” by many researchers because it primarily reflects baroreceptor activity while at rest.[56] As discussed previously, the vagus nerves are a major conduit through which afferent neurological signals from the heart are relayed to the brain, including baroreflex signals. Baroreceptors are stretch-sensitive mechanoreceptors located in the chambers of the heart and vena cavae, carotid sinuses (which contain the most sensitive mechanoreceptors) and the aortic arch. Baroreflex gain is commonly calculated as the beat-to-beat change in HR per unit of change in BP. Decreased baroreflex gain is related to aging and impaired regulatory capacity. The existence of a cardiovascular system resonance frequency, which is caused by the delay in the feedback loops in the baroreflex system, has long been established. When the cardiovascular system oscillates at this frequency, there is a distinctive high-amplitude peak in the HRV power spectrum around 0.1 hertz. Most mathematical models show that the resonance frequency of the human cardiovascular system is determined by the feedback loops between the heart and brain.[57, 58] In humans and many other mammals, the resonance frequency of the system is approximately 0.1 hertz, equivalent to a 10-second rhythm, which is also characteristic of the coherent state described earlier.
The sympathetic nervous system does not appear to have much influence in rhythms above 0.1 hertz, while the parasympathetic system can be observed to affect heart rhythms down to 0.05 hertz (20-second rhythm). Therefore, during periods of slow respiration rates, vagal activity can easily generate oscillations in the heart rhythms that cross over into the LF band.[37, 59, 60] Thus, respiratory-related efferent vagally mediated influences are particularly present in the LF band when respiration rates are below 8.5 breaths per minute/7-second periods or when an individual sighs or takes a deep breath.[60, 61]
In ambulatory 24-hour HRV recordings, it has been suggested that the LF band reflects sympathetic activity and the LF/HF ratio has been used, controversially so, to assess the balance between sympathetic and parasympathetic activity.[62-64] A number of researchers have challenged this perspective and have persuasively argued that in resting conditions, the LF band reflects baroreflex activity and not cardiac sympathetic innervation.40, 71, 96, 105-107
The perspective that the LF band reflects sympathetic activity comes from observations of 24-hour ambulatory recordings in which there are frequent sympathetic activations primarily resulting from physical activity, but also emotional reactions, which can create oscillations in the heart rhythms that cross over from the VLF band into the lower region of the LF band. In long-term ambulatory recordings, the LF band fairly approximates sympathetic activity when increased sympathetic activity occurs.[65] Unfortunately, some authors have assumed that this interpretation is also true of short-term resting recordings and have confused slower breathing-related increases in LF power with sympathetic activity, when in reality it is almost entirely vagally mediated.
Very-Low-Frequency Band
The very-low-frequency band (VLF) is the power in the HRV power spectrum range between 0.0033 and 0.04 hertz, which equates to rhythms or modulations with periods that occur between 25 and 300 seconds. Although all 24-hour clinical measures of HRV reflecting low HRV are linked with increased risk of adverse outcomes, the VLF band has stronger associations with all-cause mortality than the LF and HF bands.[24, 66-68] Low VLF power has been shown to be associated with arrhythmic death[69] and PTSD.[70] Additionally, low power in this band has been associated with high inflammation[71, 72] in a number of studies and has been correlated with low levels of testosterone, while other biochemical markers, such as those mediated by the HPA axis (e.g., cortisol), has not.[73] Longer time periods using 24-hour HRV recordings should be obtained to provide comprehensive assessment of VLF and ULF fluctuations.[74]
Historically, the physiological explanation and mechanisms involved in the generation of the VLF component have not been as well defined as the LF and HF components. This region has been largely ignored even though it is the most predictive of adverse outcomes. Long-term regulation mechanisms and ANS activity related to thermoregulation, the renin-angiotensin system and other hormonal factors appear to contribute to this band.[75, 76] Recent work by Dr. J. Andrew Armour has shed new light on the mechanisms underlying the VLF rhythm and suggests that we have to reconsider both the mechanisms and importance of this band.
This line of research began after some surprising results from a study looking at HRV in auto transplanted hearts in dogs. In auto transplants, the heart is removed and placed back in the same animal, so there is no need for anti-rejection medications. The primary purpose of the study was to determine if the autonomic nerves reinnervated the heart post-transplant. Monthly 24-hour HRV recordings were done over a one-year period on all of the dogs with auto transplanted hearts as well as control dogs. It turned out that the nerves did re-innervate, but in a way that was not accurately reflected in HRV. It was shown that the intrinsic cardiac nervous system had neuroplasticity and restructured its neural connections. The truly surprising result was that these de-innervated hearts had higher levels of HRV than the control dogs immediately post-transplant and these levels were sustained over a one-year period, including HRV, which typically is associated with respiration (Figure 8).[77] This was unexpected because in human transplant recipients, there is very little HRV.[78]
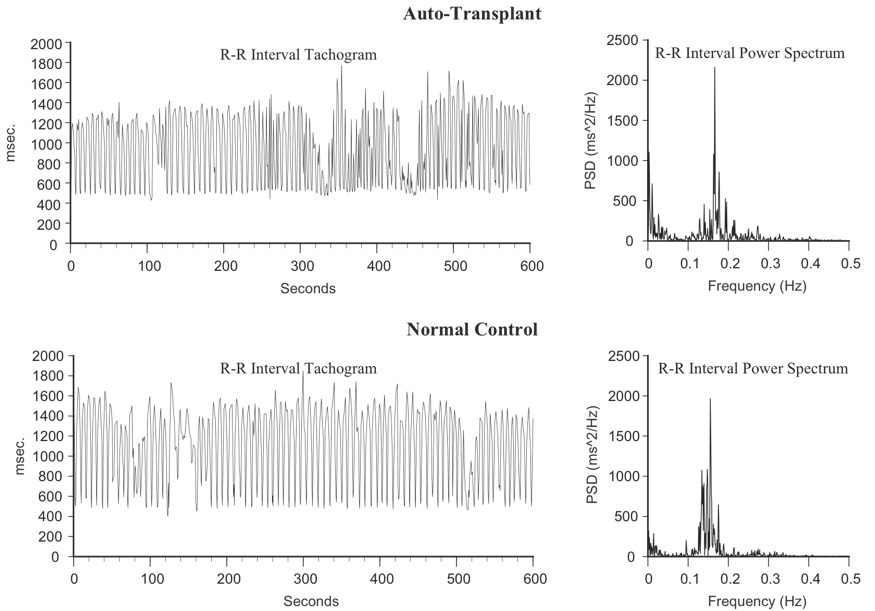
Figure 8. Heart Rhythms Generated by a Transplanted Heart At top left is the heart-rate tachogram of a dog after undergoing cardiac autotransplantation, with the accompanying top-right graph showing the HRV power spectrum. For comparison, the bottom graphs show the heart-rate tachogram and HRV power spectrum of a normal dog. Note the similarity between the two.
Following up on these results, Armour and colleagues developed methods for obtaining long-term single-neuron recordings from a beating heart and, simultaneously, from extrinsic cardiac neurons.[1] This work, combined with later findings by Kember and Armour, implies that the VLF rhythm is generated by the stimulation of afferent sensory neurons in the heart, which in turn activates various levels of the feedback and feed-forward loops in the heart’s intrinsic cardiac nervous system, as well as between the heart and neurons in the extrinsic cardiac ganglia and spinal column.[79, 80] Thus, the VLF rhythm appears to be produced by the heart itself and is an intrinsic rhythm that appears to be fundamental to health and well-being. Armour has observed that when the amplitude of the VLF rhythm at the neural level is diminished in an animal research subject, the animal is in danger and will expire shortly if the research procedures proceed. This cardiac origin of the VLF rhythm also is supported by studies showing that sympathetic blockade does not affect VLF power and VLF activity remains in tetraplegics, whose sympathetic innervation of the heart and lungs is disrupted.[81]
Circadian rhythms, core body temperature, metabolism, hormones and intrinsic rhythms generated by the heart all contribute to lower frequency rhythms (e.g., very-low-frequency and ultra-low-frequency) that extend below 0.04 hertz. In healthy individuals, there is an increase in VLF power that occurs during the night and peaks before waking.[82, 83] This increase in autonomic activity appears to correlate with the morning cortisol peak.
To summarize, experimental evidence suggests the VLF rhythm is intrinsically generated by the heart and the amplitude and frequency of these oscillations are modulated by efferent sympathetic activity. Normal VLF power appears to indicate healthy function, and increases in resting VLF power and/or shifting of frequency can reflect efferent sympathetic activity. The modulation of the frequency of this rhythm resulting from physical activity,[84] stress responses and other factors that increase efferent sympathetic activation can cause it to cross over into the lower region of the LF band during ambulatory monitoring or during short-term recordings when there is a significant emotional stressor.[5]
Time Domain Measurements of HRV
Time domain measures are the simplest to calculate and include the mean normal-to-normal (NN) intervals during the entire recording and other statistical measures such as the standard deviation between NN intervals (SDNN). However, time domain measures do not provide a means to adequately quantify autonomic dynamics or determine the rhythmic or oscillatory activity generated by the different physiological control systems.
Since they are always calculated the same way, data collected by different researchers are comparable, but only if the recording lengths are exactly the same and the data are collected under the same conditions.
Time domain indices quantify the amount of variance in the IBI using statistical measures. The three most important time domain measures are the SDNN, the SDNN index, and the RMSSD.
SDNN
The SDNN is the standard deviation of the normal (NN) sinus- initiated IBI measured in milliseconds. This measure reflects the ebb and flow of all the factors that contribute to heart rate variability (HRV). In 24-h recordings, the SDNN is highly correlated with ULF and total power.[22] In short-term resting recordings, the primary source of the variation is parasympathetically-mediated RSA, especially with slow, paced breathing protocols. Low age-adjusted values predict both morbidity and mortality. Classification within a higher SDNN category is associated with a higher probability of survival. For example, patients with moderate SDNN values, 50-100 ms, have a 400% lower risk of mortality than those with low values, 0-50 ms, in 24-h recordings.[85]
RMSSD
The RMSSD is the root mean square of successive differences between normal heartbeats. This value is obtained by first calculating each successive time difference between heartbeats in milliseconds. Then, each of the values is squared and the result is averaged before the square root of the total is obtained. The RMSSD reflects the beat-to-beat variance in heart rate and is the primary time domain measure used to estimate the vagally-mediated changes reflected in HRV. While the RMSSD is correlated with HF power.[74] Lower RMSSD values are correlated with higher scores on a risk inventory of sudden unexplained death in epilepsy.[86]
Coherence
Definitions of Coherence
Clarity of thought, speech and emotional composure
The quality of being orderly, consistent and intelligible (e.g. a coherent sentence)
Synchronization or entrainment between multiple waveforms
A constructive waveform produced by two or more waves that are phase-or frequency-locked
Order within a singular oscillatory waveform
An ordered or constructive distribution of power content within a single waveform; autocoherence (e.g. sine wave)
Many contemporary scientists believe that it is the underlying state of our physiological processes that determines the quality and stability of the feelings and emotions we experience. The feelings we label as “positive” actually reflect body states that are coherent in which “the regulation of life processes becomes efficient, or even optimal, free-flowing and easy,”[87] and where negative feelings such as anger, anxiety and frustration are examples of incoherent states. It is important to note, however, these associations are not merely metaphorical. For the brain and nervous system to function optimally, the neural activity, which encodes and distributes information, must be stable and function in a coordinated and balanced manner. The various centers within the brain must also be able to dynamically synchronize their activity in order for information to be smoothly processed and perceived. Thus, the concept of coherence is vitally important for understanding optimal function.
The various concepts and measurements embraced under the term coherence have become central to fields as diverse as quantum physics, cosmology, physiology and brain and consciousness research.[9] Coherence has several related definitions, all of which are applicable to the study of human physiology, social interactions and global affairs. The most common dictionary definition is “the quality of being logically integrated, consistent and intelligible,” as in a coherent statement.[88] A related meaning is the logical, orderly and aesthetically consistent relationship among parts.[88] Coherence always implies correlations, connectedness, consistency and efficient energy utilization. Thus, coherence refers to wholeness and global order, where the whole is greater than the sum of its individual parts.
In physics, coherence also is used to describe the coupling and degree of synchronization between different oscillating systems. In some cases, when two or more oscillatory systems operate at the same basic frequency, they can become either phase- or frequency-locked, as occurs between the photons in a laser.[89] This type of coherence is called cross-coherence and is the type of coherence that most scientists think of when they use the term. In physiology, cross-coherence occurs when two or more of the body’s oscillatory systems, such as respiration and heart rhythms, become entrained and operate at the same frequency.
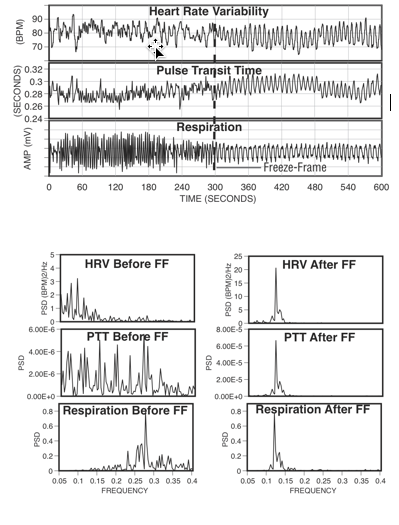
Figure 9. The top graphs show an individual’s heart rate variability, pulse transit time and respiration patterns for 10 minutes. At the 300-second mark, the individual practiced Freeze Frame (a HeartMath technique) and all three systems came into entrainment, meaning the patterns were harmonious instead of scattered and out of sync. The bottom graphs show the spectrum analysis view of the same data. The left-hand side is the spectral analysis before using Freeze Frame. Notice how each pattern looks quite different from the others. The graphs on the right show how all three systems are entrained at the same frequency after using Freeze Frame.
Another aspect of coherence relates to the dynamic rhythms produced by a single oscillatory system. The term autocoherence describes coherent activity within a single system. An ideal example is a system that exhibits sine-wavelike oscillations; the more stable the frequency, amplitude and shape, the higher the degree of coherence. When coherence is increased in a system that is coupled to other systems, it can pull the other systems into increased synchronization and more efficient function. For example, frequency pulling and entrainment can easily be seen between the heart, respiratory and blood-pressure rhythms as well as between very low frequency brain rhythms, craniosacral rhythms and electrical potentials measured across the skin.[90], [91]
Global Coherence
For any system to produce a meaningful function, it must have the property of global coherence. In humans, this includes our physical, mental, emotional and social systems. However, the energy efficiency and degree of coordinated action of any given system can vary widely and does not necessarily result in a coherent output or flow of behavior. Global coherence does not mean everyone or all parts of a system are doing the same thing simultaneously. In complex globally coherent systems, such as human beings, there is an vast amount of activity at every level of magnification or scale that spans more than two-thirds of the 73 known octaves of the electromagnetic spectrum.[92] It can appear at one level of scale that a given system is operating autonomously, yet it is perfectly coordinated within the whole. In living systems, there are microlevel systems, molecular machines, protons and electrons, organs and glands, each functioning autonomously, doing very different things at different rates, yet all working together in a complex harmoniously coordinated and synchronized manner. If this were not happening, it would be a free-for-all among the body’s independent systems, rather than a coordinated federation of interdependent systems and functions. Biologist Mae-Won Ho has suggested that coherence is the defining quality of living systems and accounts for their most characteristic properties, such as long-range order and coordination, rapid and efficient energy transfer and extreme sensitivity to specific signals.[92]
We introduced the term physiological coherence to describe the degree of order, harmony and stability in the various rhythmic activities within living systems over any given time period.[90] This harmonious order signifies a coherent system, whose efficient or optimal function is directly related to the ease and flow in life processes. In contrast, an erratic, discordant pattern of activity denotes an incoherent system whose function reflects stress and inefficient utilization of energy in life processes. Specifically, heart coherence (also referred to as cardiac coherence or resonance) can be measured by HRV analysis wherein a person’s heart-rhythm pattern becomes more ordered and sine-wavelike at a frequency of around 0.1 hertz (10 seconds).
When a person is in a more coherent state there is a shift in the relative autonomic balance toward increased parasympathetic activity (vagal tone), increased heart-brain synchronization and entrainment between diverse physiological systems. In this mode, the body’s systems function with a high degree of efficiency and harmony and natural regenerative processes are facilitated. Although physiological coherence is a natural human state that can occur spontaneously, sustained episodes generally are rare. While some rhythmic-breathing methods may induce coherence for brief periods, our research indicates that people can achieve extended periods of physiological coherence by actively self-generating positive emotions.
When functioning in a coherent mode, the heart pulls other biological oscillators into synchronization with its rhythms, thus leading to entrainment of these systems (Figure 9). Entrainment is an example of a physiological state in which there is increased coherence between multiple oscillating systems and also within each system. Thus, our findings essentially underscore what people have intuitively known for some time: Positive emotions not only “feel better,” they actually tend to increase synchronization of the body’s systems, thereby enhancing energy and enabling us to function with greater efficiency and effectiveness.
The coherence model takes a dynamic systems approach that focuses on increasing people’s self-regulatory capacity through self-management techniques that induce a physiological shift, which is reflected in the heart’s rhythms. We also suggest that rhythmic activity in living systems reflects the regulation of interconnected biological, social and environmental networks and that important biologically relevant information is encoded in the dynamic patterns of physiological activity. For example, information is encoded in the time interval between action potentials in the nervous system and patterns in the pulsatile release of hormones. Our research also suggests that the time intervals between heartbeats (HRV) also encode information, which is communicated across multiple systems and helps synchronize the system as whole. The afferent pathways from the heart and blood vessels are given more relevance in this model because of the significant degree of afferent cardiovascular input to the brain and the consistent generation of dynamic patterns generated by the heart. Our perspective is that positive emotions in general, including self-induced positive emotions, shift the entire system into a more globally coherent and harmonious physiological mode, one that is associated with improved system performance, ability to self-regulate and overall well-being. The coherence model predicts that different emotions are reflected in state-specific patterns in the heart’s rhythms [5] independent of the amount of HRV/HR (Figure 10). Recent independent work has verified this by demonstrating a 75% accuracy rate in detection of discrete emotional states from the HRV signal using a neural network approach for pattern recognition.[93] In a study of the effects of playing violent and nonviolent video games, it was found that when playing violent video games, the players had lower cardiac coherence levels and higher aggression levels than did nonviolent game players and that higher levels of coherence was negatively related to aggression.[94]
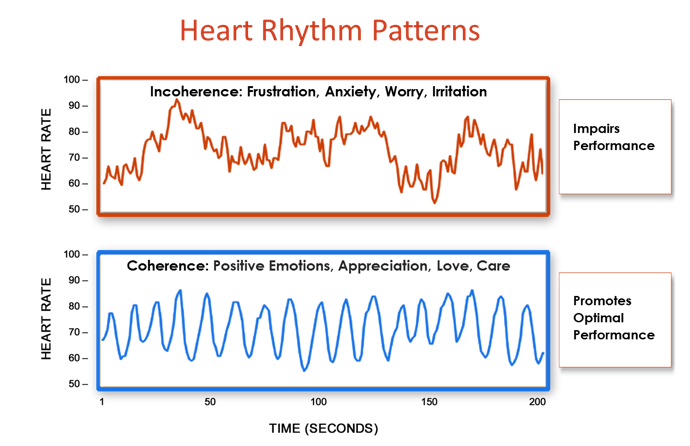
Figure 10. The shift from incoherence to coherence
The coherent state has been correlated with a general sense of well-being and improvements in cognitive, social and physical performance. We have observed this association between emotions and heart-rhythm patterns in studies conducted in both laboratory and natural settings and for both spontaneous and intentionally generated emotions.[90], [95]
Several studies in healthy subjects, which helped inform the model, show that during the experience of positive emotions, a sine-wavelike pattern naturally emerges in the heart’s rhythms without any conscious changes in breathing.[60, 96] This is likely because of more organized outputs of the subcortical structures involved in processing emotional information, as described by Pribram,[97] Porges,[7] Oppenheimer and Hopkins[43] and Thayer[15], in which the subcortical structures influence the oscillatory output of the cardiorespiratory control system in the medulla oblongata.
A brief summary of the psychophysiological coherence model is provided below. A detailed discussion on the nature of coherence can be found in two seminal articles.[5, 9]
The coherence model postulates:
- The functional status of the underlying psychophysiological system determines the range of one’s ability to adapt to challenges, self-regulate and engage in harmonious social relationships. Healthy physiological variability, feedback systems and inhibition are key elements of the complex system for maintaining stability and capacity to appropriately respond to and adapt to changing environments and social demands.
- The oscillatory activity in the heart’s rhythms reflects the status of a network of flexible relationships among dynamic interconnected neural structures in the central and autonomic nervous systems.
- State-specific emotions are reflected in the patterns of the heart’s rhythms independent of changes in the amount of heart rate variability.
- Subcortical structures constantly compare information from internal and external sensory systems via a match/mismatch process that evaluates current inputs against past experience to appraise the environment for risk or comfort and safety.
- Physiological or cardiac coherence is reflected in a more ordered sine-wavelike heart-rhythm pattern associated with increased vagally mediated HRV, entrainment between respiration, blood pressure and heart rhythms and increased synchronization between various rhythms in the EEG and cardiac cycle.
- Vagally mediated efferent HRV provides an index of the cognitive and emotional resources needed for efficient functioning in challenging environments in which delayed responding and behavioral inhibition are critical.
- Information is encoded in the time between intervals (action potentials, pulsatile release of hormones, etc.). The information contained in the interbeat intervals in the heart’s activity is communicated across multiple systems and helps synchronize the system as a whole.
- Patterns in the activity of cardiovascular afferent neuronal traffic can significantly influence cognitive performance, emotional experience and self-regulatory capacity via inputs to the thalamus, amygdala and other subcortical structures.
- Increased “rate of change” in cardiac sensory neurons (transducing BP, rhythm, etc.) during coherent states increases vagal afferent neuronal traffic, which inhibits thalamic pain pathways at the level of the spinal cord.
- Self-induced positive emotions can shift psychophysiological systems into more globally coherent and harmonious orders that are associated with improved performance and overall well-being.
The coherence model includes specific approaches for quantifying the various types of physiological coherence measures, such as cross-coherence (frequency entrainment between respiration, BP and heart rhythms), or synchronization among systems (e.g., synchronization between various EEG rhythms and the cardiac cycle), autocoherence (stability of a single waveform such as respiration or HRV patterns) and system resonance.[5] A coherent heart rhythm is defined as a relatively harmonic, sine-wavelike, signal with a very narrow, high-amplitude peak in the low frequency (LF) region of the HRV power spectrum with no major peaks in the very low frequency (VLF) or high frequency (HF) regions. Physiological coherence is assessed by identifying the maximum peak in the 0.04 to 0.26 hertz range of the HRV power spectrum, calculating the integral in a window 0.030 hertz wide, centered on the highest peak in that region and then calculating the total power of the entire spectrum. The coherence ratio is formulated as (peak power/[total power – peak power])[5].
Social Coherence
Social coherence relates to pairs, family units, groups or larger organizations in which a network of relationships exists among individuals who share common interests and objectives. Social coherence is reflected as a stable, harmonious alignment of relationships that allow for the efficient flow and utilization of energy and communication required for optimal collective cohesion and action. There are of course cycles and variations in the quality of family, team or group coherence, similar to variations in an individual’s coherence level. Coherence requires that group members are attuned and emotionally aligned and that the group’s energy is globally organized and regulated by the group as a whole. Group coherence involves the same principles of global coherence described in the introduction to this paper, but in this context it refers to the synchronized and harmonious order in the relationships between and among the individuals rather than the systems within the body. The principles, however, remain the same; in a coherent team there is freedom for the individual members to do their part and thrive while maintaining cohesion and resonance within the group’s intent and goals. Anyone who has watched a championship sports team or experienced an exceptional concert knows that something special can happen in groups that transcends their normal performance. It seems as though the players are in sync and communicating on an unseen energetic level. A growing body of evidence suggests that an energetic field is formed between individuals in groups through which communication among all the group members occurs simultaneously. In other words, there is a literal group “field” that connects all the members. Sociologist Raymond Bradley, in collaboration with eminent brain researcher, neurosurgeon and neuroscientist Dr. Karl Pribram, developed a general theory of social communication to explain the patterns of social organization common to most groups and independent of size, culture, degree of formal organization, length of existence or member characteristics. They found that most groups have a global organization and coherent network of emotional energetic relations interconnecting virtually all members into a single multilevel hierarchy.[98]
Establishing a New Baseline
From a systems perspective, the human organism is truly a vast, multidimensional information network of communicating subsystems in which mental processes, emotions and physiological systems are inextricably intertwined. Whereas our perceptions and emotions were once believed to be dictated entirely by the brain’s responses to stimuli arising from our external environment, the emerging perspectives in neuroscience more accurately describes perceptual and emotional experience as the composite of stimuli the brain receives from the external environment and the internal sensations or feedback transmitted to the brain from the bodily organs and systems.[5, 99] Thus, the heart, brain, nervous, hormonal and immune systems must all be considered fundamental components of the dynamic, interactive information network that determines our ongoing emotional experience.
Extensive work by Pribram has helped advance the understanding of the emotional system. In Pribram’s model, past experience builds within us a set of familiar patterns that are established and maintained in the neural networks. Inputs to the brain from both the external and internal environments contribute to the maintenance of these patterns.
Research has shown that the heart’s afferent neurological signals directly affect activity in the amygdala and associated nuclei, an important emotional processing center in the brain.[44] The amygdala is the key brain center that coordinates behavioral, immunological and neuroendocrine responses to environmental threats. It also serves as the processing center for emotional memory within the brain. In assessing the external environment, the amygdala scans the inputs (visual, auditory, smell) for emotional content and signals and compares them with stored emotional memories. In this way, the amygdala makes instantaneous decisions about the familiarity of incoming sensory information and because of its extensive connections to the hypothalamus and other autonomic nervous system centers is able to “hijack” the neural pathways activating the autonomic nervous system and emotional response before the higher brain centers receive the sensory information.[100]
One of the functions of the amygdala is to organize which patterns become “familiar” to the brain. If the rhythm patterns generated by the heart are disordered and incoherent, especially in early life, the amygdala learns to expect disharmony as the familiar baseline and thus we feel “at home” with incoherence, which can affect learning, creativity and emotional balance. In other words we feel “comfortable” with internal incoherence, which in this case actually is discomfort. On the basis of what has become familiar to the amygdala, the frontal cortex mediates decisions as to what constitutes appropriate behavior in any given situation. Thus, subconscious emotional memories and associated physiological patterns underlie and affect our perceptions, emotional reactions, thought processes and behavior.
From our current understanding of the elaborate feedback networks between the brain, heart and mental and emotional systems, it becomes clear that the age-old struggle between intellect and emotion will not be resolved by the mind gaining dominance over the emotions, but rather by increasing the harmonious balance between the two systems – a synthesis that provides greater access to our full range of intelligence.
Within the body, many processes and interactions occurring at different functional levels provide constant rhythmic inputs with which the brain becomes familiar. These inputs range from the rhythmic activity of the heart and our facial expressions, to digestive, respiratory and reproductive rhythms, to the constant interplay of messenger molecules produced by the cells of our body.
These inputs to the brain, translated into neural and hormonal patterns, are continuously monitored by the brain and help organize our perception, feelings and behavior. Familiar input patterns from the external environment and from within the body ultimately are written into neural circuitry and form a stable backdrop, or reference pattern, against which current and new information or experiences are compared. According to this model, when an external or internal input is sufficiently different from the familiar reference pattern, this “mismatch” or departure from the familiar underlies the generation of emotions.
The background physiological patterns with which the brain and body grow familiar are created and reinforced through life experiences and the way we perceive the world. It’s important to note that the established patterns may not necessarily be positive or healthy for a person. For example, someone living in an environment that continually triggers anger or feelings of fear is likely to become familiar with these feelings and their neural and hormonal correlates. In contrast, an individual whose experience is dominated by feelings of security, love and care likely will become familiar with the physiological patterns associated with these feelings.
In order to maintain stability and feelings of safety and comfort, we must be able to maintain a match between our current experience or “reality” and one of our previously established neural programs [101]. When we encounter a new experience or challenge, there can be a mismatch between the input patterns of the new experience and the lack of a familiar reference. Depending on the degree of mismatch, it requires either an internal adjustment (self-regulation) or an outward behavioral action to re-establish a match and feeling of comfort. When a mismatch is detected from either external or internal sensory systems, a change in activity in the central and autonomic nervous systems is produced. If the response is short-lived (one to three seconds), it is called arousal or an orienting reflex. If, however, the stimulus or event is recurrent, the brain eventually adapts and we habituate by updating the memories that serve as the reference. For example, people who live in a noisy city adapt to the ambient noise and eventually tune it out. Subsequent to this adaptation, it is only when they take a trip to the quiet countryside that the actual lack of noise seems strange and is quite noticeable. The mismatch between the familiar noisy background and the quiet setting leads to an arousal reaction that gets our attention. It is this departure from the familiar that gives rise to a signaling function that creates the experience of an emotion, alerting us to the current state of the mismatch.
In addition to the monitoring and control processes for regulation “in the here-and-now,” there are also appraisal processes that determine the degree of consistency or inconsistency between a current situation and the projected future. Appraisals of future outcomes can be broadly divided into optimistic and pessimistic [102]. Appraisals that project an inability to successfully deal with a situation may result in feelings of fear and anxiety. In keeping with the recent research on attentional bias,[103] this appraisal might not be accurate because it could be the result of hypersensitivity to cues that resemble past traumatic experiences in the current situation. Alternately, an inaccurate appraisal can caused by an instability in the neural systems, or a lack of experience or insight of how to effectively deal with the projected future situation [102]. Despite the lack of accuracy of the appraisal, the familiarity of the input can be sufficient to elicit a pessimistic response. This means we can easily get “stuck” in unhealthy emotional and behavioral patterns and lasting improvements in emotional experience or behaviors cannot be sustained in the absence of establishing a new set point for the baseline. If behavior change or improved affective states are desired, it is therefore critical to focus on strategies that help to establish a new internal reference. As we successfully navigate new situations or challenges, the positive experience updates our internal reference. In essence, we mature through this process as we learn to more effectively self-regulate our emotions and deal with new situations and challenges. It is through this process that we are able to develop a new, healthier internal baseline reference against which we match inputs so that our assessments of benign inputs are more accurate and result in feelings of safety and comfort rather than threat and anxiety.
In a study of high school students who practiced the self-regulation techniques over a three-month period, their resting HRV was significantly increased and the pattern of the HRV was significantly more coherent (Figure 11). These improvements in resting HRV coherence were significantly correlated with increased test scores and improved behaviors, suggesting that the practice of the self-regulation skills induces a more coherent heart rhythm, reinforcing the association in the sub-subcortical regulatory systems involved in a match/mismatch process between more coherent and stable rhythms in cardiovascular afferent neuronal traffic and feelings we perceive as positive [36].
By reinforcing this natural coupling in the sub-subcortical regulatory systems, the self-activation of a positive feeling can automatically initiate an increase in cardiac coherence, while at the same time; a physiological shift resulting from heart-focused breathing can help facilitate the experience of a positive emotion.
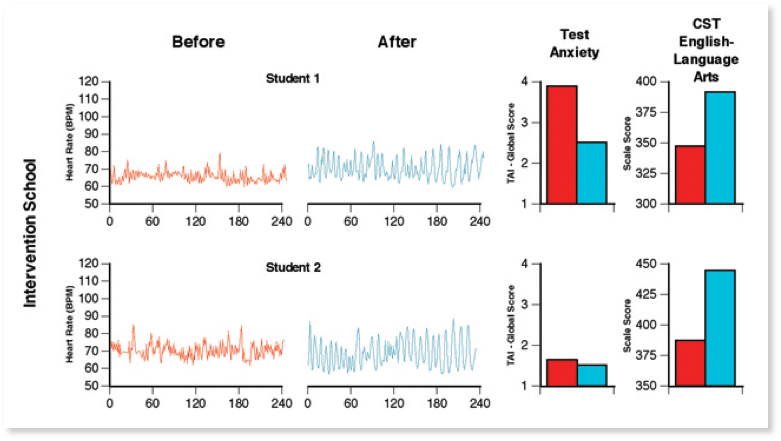
Figure 11. Typical resting state heart rate variability patterns in students. HRV recordings from the Test Edge National Demonstration Study showing examples of two students’ resting state heart rhythm patterns, both before and approximately 4 months later after the TestEdge intervention. Pre- and post-intervention test anxiety level (TAI-Global Scale score) and the CST — English Language Arts test score for each student are also shown. For the two students in the intervention school, the recordings show a shift from an erratic, irregular heart rhythm pattern (left-hand side), before the intervention, to a more coherent pattern, which indicates that the students had established a new more coherent baseline.[36]
Self-regulation and Stability
Pribram and many others have conducted numerous experiments that provide evidence that the higher brain centers that monitor the pattern-matching process can self-regulate by inhibiting or “gating” the information flowing into the brain [104]. Where we focus our attention, for example, has a powerful effect on modulating inputs and thus on determining what gets processed at higher levels. In a noisy room filled with many conversations, for instance, we have the ability to tune out the noise and focus on a single conversation of interest. In a like manner, we can modulate pain from a stubbed toe or headache or desensitize ourselves to sensations like tickling and self-direct our emotions [105]. Ultimately, when we achieve control through the process of self-regulation, it results in feelings of satisfaction and gratification. In contrast, failure to effectively self-regulate and regain control often results in feelings of frustration, impatience, anxiety, overwhelm, hopelessness or depression.
If the neural systems that maintain the baseline reference patterns are unstable, unsettled emotions and atypical reactions are likely to be experienced. These neural systems can be destabilized by trauma, stress, anxiety or chemical stimulants, to name a few possibilities. Therefore, it is clear that responding in healthy and effective ways to ongoing inner and outer demands and circumstances, such as daily life situations, depends to a great extent on the synchronization, sensitivity and stability of our physiological systems.[5, 9]
Neural inputs originate from numerous organs and muscles, especially the face. The heart and cardiovascular system, however, have far more afferent inputs than other organs and are the primary sources of consistent dynamic rhythms [3]. In addition to afferent nerve activity associated with mechanical information such as pressure and rate that occurs with each heartbeat, continuous dynamically changing patterns of afferent activity related to chemical information is sent to the brain and other systems in the body. In terms of emotional experience, there are afferent pathways to the amygdala via the nucleus of tractus solitarius and the activity in the central nucleus of the amygdala is synchronized to the cardiac cycle [106, 107]. Therefore, the afferent inputs from the cardiovascular system to the amygdala are important contributors in determining emotional experience and in establishing the set point to which the current inputs are compared.
In the context of this discussion, it is important to note that the heart’s rhythmic patterns and the patterns of afferent neurological signals change to a more ordered and stable pattern when one uses the heart-focused self-regulation techniques. Regular practice of these techniques, which include a shift of attentional focus to the center of the chest (heart area) accompanied by the conscious self-induction of a calm or positive emotional state, reinforces the association (pattern match) between a more coherent rhythm and a calm or positive emotion. Positive feelings then more automatically initiate an increase in cardiac coherence. Increased coherence initiated through heart-focused breathing tends to facilitate the felt experience of a positive emotion. Thus, practice facilitates the repatterning process. This is important in situations where there has been a sustained exposure to truly high-risk environments or trauma in the past, but which no longer are in effect and the patterns that developed in response to them no longer serve the individual in present safe environments.
Through this feed-forward process, regulatory capacity is increased and new reference patterns are established, which the system then strives to maintain, making it easier for people to maintain stability and self-directed control during daily activities, even in more challenging situations. Without a shift in the underlying baseline, it is exceedingly difficult to sustain behavioral change, placing people at risk of living their lives through the automatic filters of past familiar experience.
Self-Regulation Techniques that Reduce Stress and Enhance Human Performance
With stress levels continuing to rise around the world, people are becoming more conscious not only of the long-term effects of stress, but also of how unmanaged emotions compromise the quality of one’s day-to-day life, limiting mental clarity, productivity, adaptability to life’s challenges and enjoyment of its gifts.
While most of the adult population reports experiencing personal or emotional problems in the course of a year, about 50% of these people say that they are unable to solve their problems and about one-third state that they are unable to do anything to make their problems more bearable.
The research on heart-brain interactions has informed the development of a set of self-regulation techniques, and practices the learning of which can be supported with the use of HRV coherence feedback technologies, collectively known as the HeartMath System.[108-112] The HeartMath system offers individuals a systematic and reliable means to intentionally self-regulate and shift out of a state of emotional unease or stress into a “new” positive state of emotional calm and stability. This occurs as a result of a practice in which an individual intentionally activates a positive or calm emotional state as a future target and activates a shift in patterns of the heart’s activity to a more coherent state that enables the person to achieve and maintain stability and emotional composure.
The techniques are designed to enable people to intervene in the moment when negative and disruptive emotions are triggered, thus interrupting the body’s normal stress response and initiating a shift toward increased coherence. This shift facilitates higher cognitive functioning, intuitive access and increased emotional regulation, all of which normally are compromised during stress and negative emotional states. The shift in the pattern of the heart’s input to the brain thus serves to reinforce the self-generated positive emotional shift, making it easier to sustain.
Through consistent use of HeartMath tools, the coupling between the psychophysiological coherence mode and positive emotions is further reinforced.
Self-Regulation Techniques That Increase Coherence
There is a paradigm shift emerging on behavioral intervention approaches that teach people self-regulation strategies that include a physiological aspect such as HRV coherence feedback and that naturally increase vagal traffic. For example, there are many studies showing that the practice of breathing at 6 breaths per minute, supported by HRV feedback, induces the coherence rhythm and has a wide range of benefits.[113-119]
In addition to clinical applications, HRV coherence feedback training often is used to support self-regulation skill acquisition in educational, corporate, law enforcement and military settings. Several systems that assess the degree of coherence in the user’s heart rhythms are available. The majority of these systems use a noninvasive earlobe or finger pulse sensor and display the user’s heart rhythm to provide feedback on their level of coherence.
Emotional self-regulation strategies may contribute to improved health and performance. Alone or in combination with HRV coherence feedback training, these strategies have been shown to increase resilience and accelerate recovery from stressors or trauma.[12, 120-122] Self-induced positive emotions can initiate a shift to increased cardiac coherence without any conscious intention to change the breathing rhythm.[60, 96] Typically, when people are able to self-activate a positive or calming feeling rather than remain focused on their breathing, they enjoy the shift in feeling and are able to sustain high levels of coherence for much longer time periods.[39]
Heart-focused self-regulation techniques and assistive technologies that provide real-time HRV coherence feedback provide a systematic process for self-regulating thoughts, emotions, behaviors and increasing physiological coherence. Many of these techniques (e.g., HeartMath’s Heart-Focused Breathing™, Freeze Frame, Inner-Ease™ and Quick Coherence®[109]) are designed to enable people to intervene in the moment they start to experience stress reactions or unproductive thoughts or emotions. With practice, one is able to use any of the techniques to shift into a more coherent physiological state before, during and after challenging or adverse situations, thus optimizing mental clarity, emotional composure and stability.
The first step in most of the techniques developed by the HeartMath Institute is called Heart-Focused Breathing, which includes placing one’s attention in the center of the chest (the area of the heart) and imagining the breath is flowing in and out of the chest area while breathing a little slower and deeper than usual. Conscious regulation of one’s respiration at a 10-second rhythm (0.1 hertz) increases cardiac coherence and starts the process of shifting into a more coherent state.[5, 39] With conscious control over breathing, an individual can slow the rate and increase the depth of the breathing rhythm. This takes advantage of physiological mechanisms to modulate efferent vagal activity and thus the heart rhythm. This increases vagal afferent nerve traffic and increases the coherence (stability) in the patterns of vagal afferent nerve traffic. In turn, this influences the neural systems involved in regulating sympathetic outflow, informing emotional experience and synchronizing neural structures underlying cognitive processes.[5]
In addition to the self-regulation techniques that are primarily designed to be used in the moment, the Heart Lock-In® Technique is more appropriate when one has more time to focus on sustaining a coherent state. It enables people to “lock in” the positive feeling states associated with the heart in order to boost their energy, heighten peace and clarity and effectively retrain their physiology to sustain longer periods of coherent function. With consistent practice, the Heart Lock-In technique facilitates the establishment of new reference patterns promoting increased physiological efficiency, mental acuity and emotional stability as a new baseline or norm.
While the HeartMath tools are intentionally designed to be easily learned and used in day-to-day life, our experience working with people of diverse ages, cultures, educational backgrounds and professions suggests that these techniques often facilitate profound shifts in perception, emotion and awareness. Moreover, extensive laboratory research performed at HMI has shown that the physiological changes accompanying such shifts are dramatic.
Several studies using various combinations of these self-regulation techniques have found significant correlations between HRV coherence and improvements in cognitive function and self-regulatory capacity. For example, a study of middle school students with attention-deficit hyperactivity disorder showed a wide range of significant improvements in short- and long-term memory, ability to focus and significant improvements in behaviors both at home and in school.[34] A study of 41 fighter pilots engaging in flight simulator tasks found a significant correlation between higher levels of performance and heart-rhythm coherence as well as lower levels of frustration.[123] A study of recently returning soldiers from Iraq who were diagnosed with PTSD found that relatively brief periods of HRV coherence training combined with practicing the Quick Coherence Technique resulted in significant improvements in the ability to self-regulate along with a wide range of cognitive functions. The degree of improvement correlated with increased cardiac coherence.[35] Other studies have shown increases in parasympathetic activity (vagal tone),[60] reductions in cortisol and increases in DHEA,[42] decreases in blood pressure and stress measures in hypertensive populations,[39, 41] reduced health-care costs[38] and significant improvements in the functional capacity of patients with congestive heart failure.[124] In addition, a study of correctional officers showed reductions in systolic and diastolic BP, total cholesterol, fasting glucose, overall stress, anger, fatigue and hostility.[40] Similar results were obtained in several studies with police officers.[122, 125]
In addition to the emotional self-regulation techniques, there are other approaches that also increase HRV coherence. For example, a study of Zen monks found that monks with greater experience in meditation tended to have more coherent heart rhythms during their resting recording than those who had been monks for less than two years.[126] A study of autogenic training showed increased HRV coherence and found that cardiac coherence was strongly correlated with EEG alpha activity. The authors suggested that cardiac coherence could be a general marker for the meditative state.[127] However, this does not suggest that all meditation or prayer styles increase coherence, unless the coherence state is driven by a focus on breathing at a 10-second rhythm or the activation of a positive emotion.[128-131] For example, a study examining HRV while reciting rosary or bead prayers and yoga mantras found that a coherent rhythm was produced by rhythmically breathing but not by random verbalization or breathing. The authors ascribed the mechanisms for this finding to a breathing pattern of 6-cycles per minute.[132] In a study of the effects of five different types of prayer on HRV, it was found that all types of prayer elicited increased cardiac coherence. However, prayers of gratefulness and heartfelt love resulted in definitively higher coherence levels.[133] It also has been shown that tensing the large muscles in the legs in a rhythmical manner at a 10-second rhythm can induce a coherent heart rhythm.[134]
Bibliography
- Armour, J.A., Neurocardiology–Anatomical and functional principles, 2003, Boulder Creek, CA: HeartMath Research Center, HeartMath Institute, Publication No. 03-011.
- Armour, J.A. and J.L. Ardell, eds. Neurocardiology. 1994, Oxford University Press: New York.
- Cameron, O.G., Visceral Sensory Neuroscience: Interception, 2002, New York: Oxford University Press.
- Kukanova, B. and B. Mravec, Complex intracardiac nervous system. Bratisl Lek Listy, 2006. 107(3): p. 45-51.
- McCraty, R., Atkinson, M., Tomasino, D., & Bradley, R. T, The coherent heart: Heart-brain interactions, psychophysiological coherence, and the emergence of system-wide order. Integral Review, 2009. 5(2): p. 10-115.
- Armour, J.A., Peripheral autonomic neuronal interactions in cardiac regulation, in Neurocardiology, J.A. Armour and J.L. Ardell, Editors. 1994, Oxford University Press: New York. p. 219-244.
- Porges, S.W., The polyvagal perspective. Biol Psychol, 2007. 74(2): p. 116-43.
- Singer, D.H., et al., Low heart rate variability and sudden cardiac death. Journal of Electrocardiology, 1988(Supplemental issue): p. S46-S55.
- McCraty, R., Childre, D, Coherence: Bridging Personal, Social and Global Health. Alternative Therapies in Health and Medicine, 2010. 16(4): p. 10-24.
- Singer, D.H., High heart rate variability, marker of healthy longevity. Am J Cardiol, 2010. 106(6): p. 910.
- Geisler, F.C., et al., Cardiac vagal tone is associated with social engagement and self-regulation. Biol Psychol, 2013. 93(2): p. 279-86.
- McCraty, R. and M. Zayas, Cardiac coherence, self-regulation, autonomic stability, and psychosocial well-being. Frontiers in Psychology, 2014. 5(September ): p. 1-13.
- Reynard, A., et al., Heart rate variability as a marker of self-regulation. Appl Psychophysiol Biofeedback, 2011. 36(3): p. 209-15.
- Segerstrom, S.C. and L.S. Nes, Heart rate variability reflects self-regulatory strength, effort, and fatigue. Psychol Sci, 2007. 18(3): p. 275-81.
- Thayer, J.F., et al., Heart rate variability, prefrontal neural function, and cognitive performance: the neurovisceral integration perspective on self-regulation, adaptation, and health. Ann Behav Med, 2009. 37(2): p. 141-53.
- Camm, A.J., et al., Heart rate variability standards of measurement, physiological interpretation, and clinical use. Task Force of the European Society of Cardiology and the North American Society of Pacing and Electrophysiology. Circulation, 1996. 93(5): p. 1043-1065.
- Hon, E.H. and S.T. Lee, Electronic evaluations of the fetal heart rate patterns preceeding fetal death: further observations. American Journal of Obstetric Gynecology, 1965. 87: p. 814-826.
- Braune, H.J. and U. Geisendorfer, Measurement of heart rate variations: influencing factors, normal values and diagnostic impact on diabetic autonomic neuropathy. Diabetes Res Clin Pract, 1995. 29(3): p. 179-87.
- Vinik, A.I., et al., Diabetic autonomic neuropathy. Diabetes Care, 2003. 26(5): p. 1553-79.
- Ewing, D., I. Campbell, and B. Clarke, Mortality in diabetic autonomic neuropathy. Lancet, 1976. 1: p. 601-603.
- Wolf, M.M., et al., Sinus arrhythmia in acute mycardial infarction. Medical Journal of Australia, 1978. 2: p. 52-53.
- Umetani, K., et al., Twenty-four hour time domain heart rate variability and heart rate: relations to age and gender over nine decades. J Am Coll Cardiol, 1998. 31(3): p. 593-601.
- Dekker, J.M., et al., Heart rate variability from short electrocardiographic recordings predicts mortality from all causes in middle-aged and elderly men. The Zutphen Study. American Journal of Epidemiology, 1997. 145(10): p. 899-908.
- Tsuji, H., et al., Reduced heart rate variability and mortality risk in an elderly cohort. The Framingham Heart Study. Circulation, 1994. 90(2): p. 878-883.
- Berntson, G.G., et al., Cardiac autonomic balance versus cardiac regulatory capacity. Psychophysiology, 2008. 45(4): p. 643-52.
- Beauchaine, T., Vagal tone, development, and Gray’s motivational theory: toward an integrated model of autonomic nervous system functioning in psychopathology. Dev Psychopathol, 2001. 13(2): p. 183-214.
- Geisler, F. and T. Kubiak, Heart rate variability predicts self‐control in goal pursuit. European Journal of Personality, 2009. 23(8): p. 623-633.
- Appelhans, B. and L. Luecken, Heart Rate Variability as an Index of Regulated Emotional Responding. Review of General Psychology, 2006. 10(3): p. 229-240.
- Geisler, F., et al., The impact of heart rate variability on subjective well-being is mediated by emotion regulation. Personality and Individual Differences, 2010. 49(7): p. 723-728.
- Smith, T.W., et al., Matters of the variable heart: respiratory sinus arrhythmia response to marital interaction and associations with marital quality. J Pers Soc Psychol, 2011. 100(1): p. 103-19.
- Nasermoaddeli, A., M. Sekine, and S. Kagamimori, Association between sense of coherence and heart rate variability in healthy subjects. Environ Health Prev Med, 2004. 9(6): p. 272-4.
- Zohar, A., R. Cloninger, and R. McCraty, Personality and Heart Rate Variability: Exploring Pathways from Personality to Cardiac Coherence and Health. Open Journal of Social Sciences, 2013. 1(6): p. 32-39.
- Ramaekers, D., et al., Association between cardiac autonomic function and coping style in healthy subjects. Pacing Clin Electrophysiol, 1998. 21(8): p. 1546-52.
- Lloyd, A., Brett, D., Wesnes, K., Coherence Training Improves Cognitive Functions and Behavior In Children with ADHD. Alternative Therapies in Health and Medicine, 2010. 16(4): p. 34-42.
- Ginsberg, J.P., Berry, M.E., Powell, D.A., Cardiac Coherence and PTSD in Combat Veterans. Alternative Therapies in Health and Medicine, 2010. 16(4): p. 52-60.
- Bradley, R.T., et al., Emotion self-regulation, psychophysiological coherence, and test anxiety: results from an experiment using electrophysiological measures. Appl Psychophysiol Biofeedback, 2010. 35(4): p. 261-83.
- Lehrer, P.M., et al., Heart rate variability biofeedback increases baroreflex gain and peak expiratory flow. Psychosomatic Medicine, 2003. 65(5): p. 796-805.
- Bedell, W., Coherence and hearlth care cost – RCA acturial study: A cost-effectivness cohort study Alternative Therapies in Health and Medicine, 2010. 16(4): p. 26-31.
- Alabdulgader, A., Coherence: A Novel Nonpharmacological Modality for Lowering Blood Pressure in Hypertensive Patients. Global Advances in Health and Medicne, 2012. 1(2): p. 54-62.
- McCraty, R., et al., New hope for correctional officers: an innovative program for reducing stress and health risks. Appl Psychophysiol Biofeedback, 2009. 34(4): p. 251-72.
- McCraty, R., M. Atkinson, and D. Tomasino, Impact of a workplace stress reduction program on blood pressure and emotional health in hypertensive employees. J Altern Complement Med, 2003. 9(3): p. 355-69.
- McCraty, R., et al., The impact of a new emotional self-management program on stress, emotions, heart rate variability, DHEA and cortisol. Integr Physiol Behav Sci, 1998. 33(2): p. 151-70.
- Oppenheimer, S. and D. Hopkins, Suprabulbar neuronal regulation of the heart, in Neurocardiology, J.A. Armour and J.L. Ardell, Editors. 1994, Oxford University Press: New York. p. 309-341.
- Hopkins, D. and H. Ellenberger, Cardiorespiratory neurons in the mudulla oblongata: Input and output relationhsips, in Neurocardiology, J.A. Armour and J.L. Ardell, Editors. 1994, Oxford University Press: New York. p. 219-244.
- Armour, J.A., Anatomy and function of the intrathoracic neurons regulating the mammalian heart, in Reflex Control of the Circulation, I.H. Zucker and J.P. Gilmore, Editors. 1991, CRC Press: Boca Raton. p. 1-37.
- Beaumont, E., et al., Network interactions within the canine intrinsic cardiac nervous system: implications for reflex control of regional cardiac function. J Physiol, 2013. 591(Pt 18): p. 4515-33.
- Hainsworth, R., The control and physiological importance on heart rate, in Heart Rate Variability, M. Malik and A.J. Camm, Editors. 1995, Futura Publishing COmpany, Inc.: Armonk NY. p. 3-19.
- Palatini, P., Elevated heart rate as a predictor of increased cardiovascular morbidity. J Hypertens Suppl, 1999. 17(3): p. S3-10.
- Stampfer, H.G., The relationship between psychiatric illness and the circadian pattern of heart rate. Aust N Z J Psychiatry, 1998. 32(2): p. 187-98.
- Stampfer, H.G. and S.B. Dimmitt, Variations in circadian heart rate in psychiatric disorders: theoretical and practical implications. ChronoPhysiology and Therapy, 2013. 3: p. 41–50.
- Opthof, T., The normal range and determinants of the intrinsic heart rate in man. Cardiovasc Res, 2000. 45(1): p. 177-84.
- Umetani, K., C.L. Duda, and D.H. Singer. Aging effects on cycle length dependence of heart rate variability. in Biomedical Engineering Conference, 1996. 1996. Proceedings of the 1996 Fifteenth Southern. IEEE.
- Electrophysiology, T.F.o.t.E.S.o.C.a.t.N.A.S.o.P.a., Heart rate variability: Standards of measurement, physiological interpretation, and clinical use. Circulation, 1996. 93: p. 1043-1065.
- Hirsch, J.A. and B. Bishop, Respiratory sinus arrhythmia in humans: How breathing pattern modulates heart rate. American Journal of Physiology, 1981. 241(4): p. H620-H629.
- Eckberg, D.L., Human sinus arrhythmia as an index of vagal outflow. Journal of Applied Physiology, 1983. 54: p. 961-966.
- Malliani, A., Association of Heart Rate Variability components with physiological regulatory mechanisms, in Heart Rate Variability, M. Malik and A.J. Camm, Editors. 1995, Futura Publishing COmpany, Inc.: Armonk NY. p. 173-188.
- deBoer, R.W., J.M. Karemaker, and J. Strackee, Hemodynamic fluctuations and baroreflex sensitivity in humans: a beat-to-beat model. Am J Physiol, 1987. 253(3 Pt 2): p. H680-9.
- Baselli, G., et al., Model for the assessment of heart period variability interactions of respiration influences. Medical and Biological Engineering and Computing, 1994. 32(2): p. 143-152.
- Ahmed, A.K., J.B. Harness, and A.J. Mearns, Respiratory Control of Heart Rate. Eur J Appl Physiol, 1982. 50: p. 95-104.
- Tiller, W.A., R. McCraty, and M. Atkinson, Cardiac coherence: a new, noninvasive measure of autonomic nervous system order. Altern Ther Health Med, 1996. 2(1): p. 52-65.
- Brown, T.E., et al., Important influence of respiration on human R-R interval power spectra is largely ignored. J Appl Physiol (1985), 1993. 75(5): p. 2310-7.
- Malliani, A., et al., Power spectral analysis of cardiovascular variability in patients at risk for sudden cardiac death. J Cardiovasc Electrophysiol, 1994. 5(3): p. 274-86.
- Pal, G.K., et al., Sympathovagal imbalance contributes to prehypertension status and cardiovascular risks attributed by insulin resistance, inflammation, dyslipidemia and oxidative stress in first degree relatives of type 2 diabetics. PLoS One, 2013. 8(11): p. e78072.
- Pagani, M., F. Lombardi, and S. Guzzette, Power spectral analysis of heart rate and arterial pressure variabilities as a marker of sympatho-vagal interaction in man and conscious dog. Circulation Research, 1986. 59: p. 178-184.
- Axelrod, S., et al., Spectral analysis of fluctuations in heart rate: An objective evaluation. Nephron, 1987. 45: p. 202-206.
- Schmidt, H., et al., Autonomic dysfunction predicts mortality in patients with multiple organ dysfunction syndrome of different age groups. Crit Care Med, 2005. 33(9): p. 1994-2002.
- Hadase, M., et al., Very low frequency power of heart rate variability is a powerful predictor of clinical prognosis in patients with congestive heart failure. Circ J, 2004. 68(4): p. 343-7.
- Tsuji, H., et al., Impact of reduced heart rate variability on risk for cardiac events. The Framingham Heart Study. Circulation, 1996. 94(11): p. 2850-5.
- Bigger, J.T., Jr., et al., Frequency domain measures of heart period variability and mortality after myocardial infarction. Circulation, 1992. 85(1): p. 164-71.
- Shah, A.J., et al., Posttraumatic stress disorder and impaired autonomic modulation in male twins. Biol Psychiatry, 2013. 73(11): p. 1103-10.
- Lampert, R., et al., Decreased heart rate variability is associated with higher levels of inflammation in middle-aged men. Am Heart J, 2008. 156(4): p. 759 e1-7.
- Carney, R.M., et al., Heart rate variability and markers of inflammation and coagulation in depressed patients with coronary heart disease. J Psychosom Res, 2007. 62(4): p. 463-7.
- Theorell, T., et al., Saliva testosterone and heart rate variability in the professional symphony orchestra after “public faintings” of an orchestra member. Psychoneuroendocrinology, 2007. 32(6): p. 660-8.
- Kleiger, R.E., P.K. Stein, and J.T. Bigger, Jr., Heart rate variability: measurement and clinical utility. Ann Noninvasive Electrocardiol, 2005. 10(1): p. 88-101.
- Akselrod, S., et al., Power spectrum analysis of heart rate fluctuation: a quantitative probe of beat-to-beat cardiovascular control. Science, 1981. 213(10): p. 220-222.
- Cerutti, S., A.M. Bianchi, and L.T. Mainardi, Spectral analysis of the heart rate variability signal, in Heart Rate Variability, M. Malik and A.J. Camm, Editors. 1995, Futura Publishing COmpany, Inc.: Armonk NY. p. 63-74.
- Murphy, D.A., et al., The heart reinnervates after transplantation. Ann Thorac Surg, 2000. 69(6): p. 1769-81.
- Ramaekers, D., et al., Heart rate variability after cardiac transplantation in humans. Pacing Clin Electrophysiol, 1996. 19(12 Pt 1): p. 2112-9.
- Kember, G., et al., Competition model for aperiodic stochastic resonance in a Fitzhugh-Nagumo model of cardiac sensory neurons. Physical Review E, 2001. 63(4 Pt 1): p. 041911.
- Kember, G.C., et al., Aperiodic stochastic resonance in a hysteretic population of cardiac neurons. Physical Review E, 2000. 61(2): p. 1816-1824.
- Berntson, G.G., et al., Heart rate variability: origins, methods, and interpretive caveats. Psychophysiology, 1997. 34(6): p. 623-48.
- Huikuri, H.V., et al., Circadian rhythms of frequency domain measures of heart rate variability in healthy subjects and patients with coronary artery disease. Effects of arousal and upright posture. Circulation, 1994. 90(1): p. 121-6.
- Singh, R.B., et al., Circadian heart rate and blood pressure variability considered for research and patient care. Int J Cardiol, 2003. 87(1): p. 9-28; discussion 29-30.
- Bernardi, L., et al., Physical activity influences heart rate variability and very-low-frequency components in Holter electrocardiograms. Cardiovasc Res, 1996. 32(2): p. 234-7.
- Kleiger, R.E., et al., Decreased heart rate variability and its association with increased mortality after acute myocardial infarction. American Journal of Cardiology, 1987. 59(4): p. 256-262.
- DeGiorgio, C.M., et al., RMSSD, a measure of vagus-mediated heart rate variability, is associated with risk factors for SUDEP: the SUDEP-7 Inventory. Epilepsy Behav, 2010. 19(1): p. 78-81.
- Damasio, A., Looking for Spinoza: Joy, Sorrow, and the Feeling Brain. 2003, Orlando: Harcourt.
- Stein, J., ed. The Random House College Dictionary. 1975, Random House: New York. 261.
- Strogatz, S. and I. Stewart, Coupled Oscillators and Biological Synchronization. Scientific American, 1993(December): p. 102-109.
- Tiller, W.A., R. McCraty, and M. Atkinson, Cardiac coherence: A new, noninvasive measure of autonomic nervous system order. Alternative Therapies in Health and Medicine, 1996. 2(1): p. 52-65.
- Bradley, R.T. and K.H. Pribram, Communication and stability in social collectives. Journal of Social and Evolutionary Systems, 1998. 21(1): p. 29-80.
- Ho, M.-W., The Rainbow and the Worm: The Physics of Organisms. 2005, Singapore: World Scientific Publishing Co.
- Leon, E., et al., Affect-aware behavior modelling and control inside an intelligent environment Pervasive and Mobile Computing doi:10.1016/j.pmcj.2009.12.002, 2010.
- Hasan, Y., L. Begue, and B.J. Bushman, Violent video games stress people out and make them more aggressive. Aggress Behav, 2013. 39(1): p. 64-70.
- McCraty, R., et al., The effects of emotions on short-term power spectrum analysis of heart rate variability. American Journal of Cardiology, 1995. 76(14): p. 1089-1093.
- McCraty, R., et al., The effects of emotions on short-term power spectrum analysis of heart rate variability. Am J Cardiol, 1995. 76(14): p. 1089-93.
- Pribram, K.H. and F.T. Melges, Psychophysiological basis of emotion, in Handbook of Clinical Neurology, P.J. Vinken and G.W. Bruyn, Editors. 1969, North-Holland Publishing Company: Amsterdam. p. 316-341.
- Bradley, R.T., Charisma and Social Structure: A Study of Love and Power, Wholeness and Transformation. 1987, New York: Paragon House.
- Damasio, A.R., Descartes’ Error: Emotion, Reason and the Human Brain. 1994, New York: G.P. Putnam’s Sons.
- LeDoux, J., The Emotional Brain: The Mysterious Underpinnings of Emotional Life. 1996, New York: Simon and Schuster.
- Miller, G.A., E.H. Galanter, and K.H. Pribram, Plans and the Structure of Behavior. 1960, New York: Henry Holt & Co.v
- Pribram, K.H., Feelings as monitors, in Feelings and Emotions, M.B. Arnold, Editor 1970, Academic Press: New York. p. 41-53.
- Olatunji, B.O., et al., Heightened attentional capture by threat in veterans with PTSD. J Abnorm Psychol, 2013. 122(2): p. 397-405.
- Pribram, K.H. and D. McGuinness, Arousal, activation, and effort in the control of attention. Psychological Review, 1975. 82(2): p. 116-149.
- Pribram, K.H., Languages of the Brain: Experimental Paradoxes and Principals in Neuropsychology. 1971, New York: Brandon House.
- Zhang, J.X., R.M. Harper, and R.C. Frysinger, Respiratory modulation of neuronal discharge in the central nucleus of the amygdala during sleep and waking states. Experimental Neurology, 1986. 91: p. 193-207.
- Frysinger, R.C. and R.M. Harper, Cardiac and respiratory correlations with unit discharge in epileptic human temporal lobe. Epilepsia, 1990. 31(2): p. 162-171.
- Childre, D.L., Freeze-Frame®, Fast Action Stress Relief. 1994, Boulder Creek: Planetary Publications.
- Childre, D. and H. Martin, The HeartMath Solution. 1999, San Francisco: HarperSanFrancisco.
- Childre, D. and B. Cryer, From Chaos to Coherence: The Power to Change Performance. 2000, Boulder Creek, CA: Planetary.
- Childre, D. and D. Rozman, Overcoming Emotional Chaos: Eliminate Anxiety, Lift Depression and Create Security in Your Life. 2002, San Diego: Jodere Group.
- Childre, D. and D. Rozman, Transforming Stress: The HeartMath Solution to Relieving Worry, Fatigue, and Tension. 2005, Oakland, CA: New Harbinger Publications.
- Lehrer, P., et al., Heart rate variability biofeedback: effects of age on heart rate variability, baroreflex gain, and asthma. Chest, 2006. 129(2): p. 278-84.
- Ratanasiripong, P., N. Ratanasiripong, and D. Kathalae, Biofeedback Intervention for Stress and Anxiety among Nursing Students: A Randomized Controlled Trial. International Scholarly Research Network Nurs. 2012;2012:827972, 2012.
- Beckham, A.J., T.B. Greene, and S. Meltzer-Brody, A pilot study of heart rate variability biofeedback therapy in the treatment of perinatal depression on a specialized perinatal psychiatry inpatient unit. Arch Womens Ment Health, 2013. 16(1): p. 59-65.
- Siepmann, M., et al., A pilot study on the effects of heart rate variability biofeedback in patients with depression and in healthy subjects. Appl Psychophysiol Biofeedback, 2008. 33(4): p. 195-201.
- Hallman, D.M., et al., Effects of heart rate variability biofeedback in subjects with stress-related chronic neck pain: a pilot study. Appl Psychophysiol Biofeedback, 2011. 36(2): p. 71-80.
- Henriques, G., et al., Exploring the effectiveness of a computer-based heart rate variability biofeedback program in reducing anxiety in college students. Appl Psychophysiol Biofeedback, 2011. 36(2): p. 101-12.
- Lin, G., et al., Heart rate variability biofeedback decreases blood pressure in prehypertensive subjects by improving autonomic function and baroreflex. J Altern Complement Med, 2012. 18(2): p. 143-52.
- McCraty, R. and D. Tomasino, Coherence-building techniques and heart rhythm coherence feedback: New tools for stress reduction, disease prevention, and rehabilitation, in Clinical Psychology and Heart Disease, E. Molinari, A. Compare, and G. Parati, Editors. 2006, Springer-Verlag: Milan, Italy.
- McCraty, R. and D. Childre, Coherence: bridging personal, social, and global health. Altern Ther Health Med, 2010. 16(4): p. 10-24.
- McCraty, R. and M. Atkinson, Resilence Training Program Reduces Physiological and Psychological Stress in Police Officers. Global Advances in Health and Medicne, 2012. 1(5): p. 44-66.
- Li, W.-C., et al., The Investigation of Visual Attention and Workload by Experts and Novices in the Cockpit, in Engineering Psychology and Cognitive Ergonomics. Applications and Services, D. Harris, Editor 2013, Springer Berlin Heidelberg. p. 167-176.
- Luskin, F., K. Newell, and W. Haskell, Stress management training of elderly patients with congestive heart failure: pilot study. Preventive Cardiology, 1999. 2: p. 101-104.
- Weltman, G., et al., Police Department Personnel Stress Resilience Training: An Institutional Case Study. Global Advances in Health and Medicne, 2014. 3(2): p. 72-79.
- Lehrer, P., Y. Sasaki, and Y. Saito, Zazen and cardiac variability. Psychosomatic Medicine, 1999. 61: p. 812-821.
- Kim, D.-K., et al., Dynamic correlations between heart and brain rhythm during Autogenic meditation. Front. Hum. Neurosci., 2013. 7:414.
- Peng, C.K., et al., Exaggerated heart rate oscillations during two meditation techniques. Int J Cardiol, 1999. 70(2): p. 101-7.
- Wu, S.D. and P.C. Lo, Inward-attention meditation increases parasympathetic activity: a study based on heart rate variability. Biomed Res, 2008. 29(5): p. 245-50.
- Phongsuphap, S. and Y. Pongsupap, Analysis of Heart Rate Variability during Meditation by a Pattern Recognition Method Computing in Cardiology, 2011. 38: p. 197-200.
- Stanley, R., Types or prayer, heart rate variablity and innate healing Zygon, 2009. 44(4): p. 825-846.
- Bernardi, L., et al., Effect of rosary prayer and yoga mantras on autonomic cardiovascular rhythms: Comparative study. BMJ, 2001. 323: p. 1446-1449.
- Stanley, R., Types of Prayer, Heart Rate Variablity and Innate Healing Zygon 2009. 44(4).
- Lehrer, P., et al., Effects of rhythmical muscle tension at 0.1Hz on cardiovascular resonance and the baroreflex. Biol Psychol, 2009. 81(1): p. 24-30.
All rights reserved. No part of this book may be translated or reproduced in any form without the written permission of the HeartMath Institute.
HeartMath Freeze Frame, and Heart Lock-In, are registered trademarks of the HeartMath Institute. Heart-Focused Breathing is a trademark of Doc Childre.